Hello everyone,
We've finally made it to the end, and we are just about ready to discuss biocompatibility.
There's still the matter of one last area to discuss, and I wasn't entirely sure how to go about it. I intended to focus more heavily on the immune response and acute inflammation. But, after some thought, I felt that it would be better to discuss them to a lesser extent and instead focus on the process of wound healing—though the inflammatory response will still make an appearance. Specifically, we will be looking at the difference between tissue repair and tissue regeneration, the latter being facilitated by an acellular dermal matrix, which is the same category of material that the decellularized extracellular matrix (ECM) scaffolds Foregen is using.
By comparing these two examples, I hope to demonstrate how our proposed regenerative therapy, and others like it, essentially works on a more macroscale. I strongly recommend reading the first three entries of this series before reading this one. However, it won't be invoking any complicated mathematics like the previous one. Still, it does build upon everything that I went over before. It will also provide a detailed account of what went on in our rat model, what is expected to be observed in the sheep model, and finally, in humans.
And then, following this, we will finally discuss biocompatibility.
Acute Inflammation
According to the principle of sufficient reason, it is said that everything that is exists in the way that it does due to some prior cause [4:34–50]. If I hold my pen and let go of it, we will see it fall to the floor. If I pick it up and let go again, we will see it fall again—we see the causal relation. Before we can consider wound healing, we need to consider the causal chain of events that produces such a process; for a wound to be healed, there must first be damage inflicted.
When tissue is first injured or infected, it often becomes inflamed, which is to say that it exhibits the four cardinal signs of inflammation, redness (rubor), swelling (tumor), tissue heating (calore), and pain (dolore). This is initiated by a cascade of inflammatory mediators (Table 1) released from the resulting tissue damage into the body.

These mediators trigger a cascade of physiological processes outlined in Figure 1. First, blood vessels will vasodilate, or expand, which is one of the reasons for tissue heating and redness. Capillaries in the vicinity also become more permeable, leading to fluid leakage into the interstitial space. As a result, some blood clotting proteins, like fibrinogen, enter the interstitial space, begin to clot, and form a physical barrier to wall off the injured area to keep out invading microbes. Through released chemoattractants (like I mentioned in the previous segment), immune cells are directed towards the injury site and begin to clean up debris and foreign organisms. Lastly, pain is often the by-product of releasing certain substances called kinins from the blood clotting process [5:372–373]. I will point out that acute inflammation should be distinguished from chronic inflammation. Still, for this article, we won't discuss that.

The Four Types of Resolution
After the termination of the inflammatory response (which we won't touch on), the new equilibrium state is said to be a resolution of the response above. Four possible resolutions can occur: extrusion, resorption, integration, and encapsulation. Extrusion is something that most of us have already experienced. If you ever get a splinter that you cannot remove, the body will push it out over time if you leave it alone. Resorption is the most important for Foregen's work. This is the resolution when a biodegradable material is implanted and no fibrous capsule forms. When it comes to things like orthopedic implants, integration is the desired resolution, as no fibrous capsule is formed, and the host tissue, such as bone, has integrated into the implanted material. Lastly, encapsulation is the traditional response of non-resorbable materials, which typically results in the formation of fibrous scar tissue and is characteristic of normal wound healing [6:390].
Wound Healing: Repair vs. Regeneration
Let's now look at how everything we've covered in this series fits together with an example and compare tissue repair to tissue regeneration. Let us consider a hypothetical skin wound that extends deep into the dermal layer (Figure 2). The primary means of wound healing is repair. First, we will look at how the body will usually repair this injury. Then we will look at how introducing a biomaterial scaffold (an acellular dermal matrix, to be exact) facilitates regeneration.

Skin Repair
The normal wound healing process is complex, with many moving parts that would be impossible to describe in an article such as this fully. Nevertheless, it can be broken down into four distinct stages, which often overlap:
- Hemostasis: This term means the stopping of blood flow. This would be when an injury has clotted and stopped bleeding in wound healing. In response to the injury, inflammatory triggers are released and activate inflammation.
- Inflammation: We'll discuss this in more detail, but initially, immune cells called neutrophils arrive at the wound site and begin cleaning bacteria and other foreign materials. Subsequently, another type of immune cell called macrophages replaces the neutrophils, which continue cleaning and start transitioning out of inflammation by releasing growth factors and other mediators that promote proliferation.
- Proliferation: New blood vessels begin to form (angiogenesis), fibroblasts invade the wound site, produce new ECM, and degrade the existing matrix. Keratinocytes at the wound edge migrate across the bed, resulting in reepithelialization.
- Remodeling: After the wound has been closed, tissue remodeling occurs over the following months or years. We see an accumulation of collagen and the formation of fibrous scar tissue within the defect.
This represents the completion of tissue repair. The resulting scar tissue is avascular and often has different mechanical properties from normal skin. But, the resulting tissue is functionally adequate for wound repair, where the main objective is ultimately survival [2:126, 6:392].
Skin Regeneration
The last example is how the body heals wounds of this type usually. Its primary concern is sealing off the wound to prevent fluid loss and infection and to repair the injury quickly; this is ultimately an evolutionary advantage that mammals have developed, which sets us apart from our very distant reptilian and amphibian relatives, who have much greater regenerative capabilities [1].
Adult humans carry limited regenerative capabilities, such as in the epidermal layer of skin, which is why less severe wounds don't scar. Understanding the physiological mechanisms involved in wound healing, combined with biomaterials science, allows tissue engineers to exploit normal human biology and induce tissue and organ regeneration [11].
Let us again consider the injury I Figure 2. However, this time, let's introduce a material that "fills in" the damaged dermal tissue. This hypothetical material is a scaffolding material that contains bioactive components that promote cell migration and wound healing. For this example, this is called an acellular dermal matrix, the same material that our decellularized foreskin ECMs are. Once the material is applied to a wound (Figure 3), the healing process undergoes a more controlled process. A different sequence of events happens, which results in regeneration instead of mere repair.
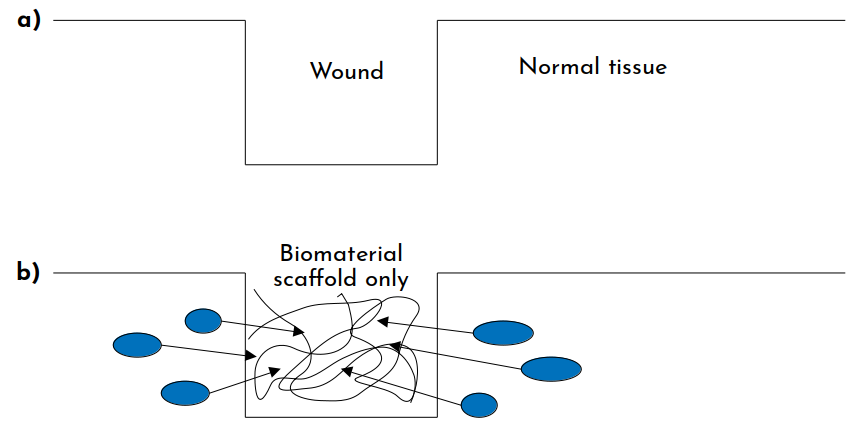
The ultimate explanation for why tissue engineering (TE) scaffolds of this kind allow for regeneration is because they bind and permit normal matrix-cell interactions, just as normal tissue does. These scaffolds are composed of (ECM), such as collagen, hyaluronic acid, or fibronectin, which promote resorption. When one of these matrices is implanted, the following series of events occur:
- Imbibition: Fluid from the wound that contains fibrin and red blood cells permeates the matrix due to its porous nature. Fibrin causes the scaffold to adhere to the injury.
- Fibroblast migration: Growth factors embedded in the matrix stimulate host cells. Immune cells begin to infiltrate the matrix, which secrete biochemical signals that promote fibroblast proliferation. The fibroblasts then migrate into the scaffold, using the collagen fibers as a scaffold, binding specifically to the adhesion molecules.
- Neovascularization: After roughly three weeks after implantation, endothelial cells begin migrating and begin lumen formation to support newly settled fibroblasts. Neovascularization is usually established by week 4.
- Matrix remodeling and maturation: Finally, the implanted scaffold is resorbed and degraded and is replaced with newly deposited collagen and ECM fibers, resulting in regenerated tissue [2:130–131].
Further proliferation and matrix production restore the tissue to its original thickness with time. The resulting neotissue has the same structure and properties as the original tissue before injury [6:398]. Because of the controlled nature of healing, we find no scar tissue formation and regenerated tissue instead.
Admittedly, this is a very generalized description, and depending on the application, the process may differ somewhat.
Biocompatibility
We are now at the finale and can adequately discuss biocompatibility. As I mentioned in the first entry, the term biocompatibility is routinely misused; even by seasoned researchers and other experts [10]. That said, if I have done my job correctly, and you have read the previous three articles, and up to this point, you will walk away from this with a solid understanding of what this concept actually is.
So, the question that all of this has been leading up to: what is biocompatibility? It isn't easy to adequately define, but, just as I did in the first article on biomaterials, I will quote what my undergraduate biomaterials textbook says:
The ability of a material to perform with an appropriate host response in a specific application. [7:2]
I imagine that this is likely how everyone of you would define the term based on its name. I have many good things to say about this text, but I have an issue in this case. This definition is not necessarily wrong, but it is easy to misinterpret this concept entirely by the way it is defined.
I want to make this as straightforward as possible: there is no such thing as a biocompatible material. There are no "biocompatible polymers" or anything of the like. Suppose someone uses biocompatible as an adjective to describe a material. In that case, it's entirely clear that they do not understand this concept. In truth, biocompatibility is a characteristic of the material-biological host system, not a material property; there is no such thing as a biocompatible material [10].
Materials affect different biological systems in different ways; how a material interacts in one place may be entirely different and distinct than how it interacts in another; just because a material in one configuration is suitable for one application does not necessarily mean using it elsewhere will be safe or produce success. Biological systems are immensely complicated, and these interactions are time-dependent. Some materials may behave differently—perhaps undesirably—after long periods of time [9].
The simple example I like to use is the following: hydroxyapatite is a ceramic material commonly used in bone tissue engineering. It works well in those applications, but I'd venture to say that if you used it to repair a cardiac defect, you would not have anything approaching good results.
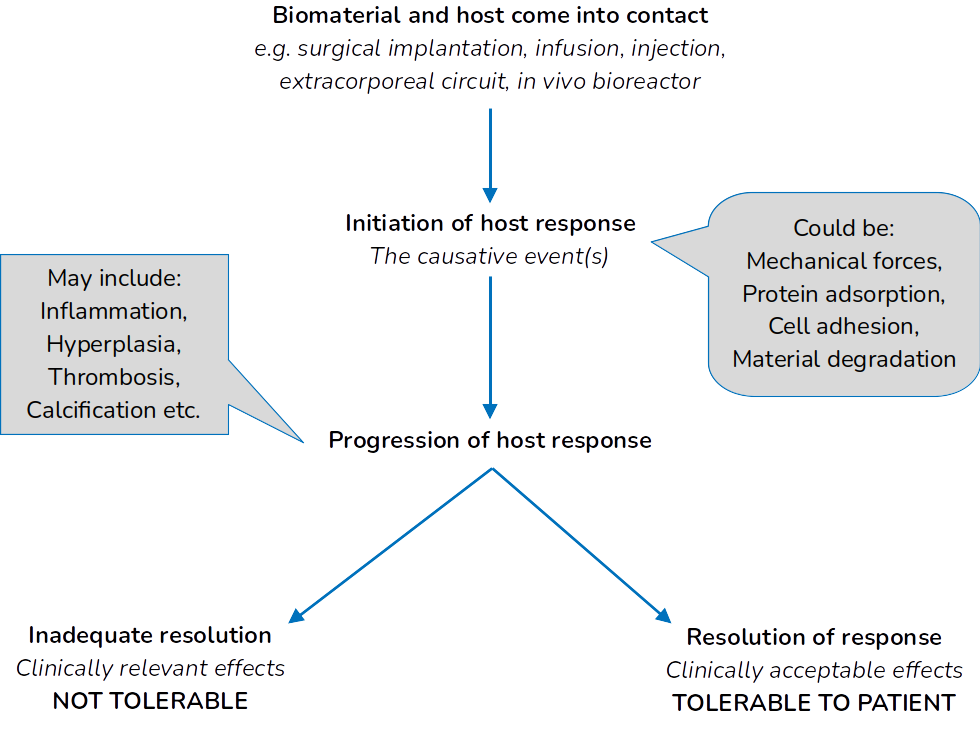
Considerations surrounding biocompatibility take many different forms depending on the area of biomedical engineering that we're considering. I will keep things within the realm of tissue engineering, though, which is ultimately based on the essential material specifications, where it is implanted, and the desired response.
Let's explore this through the lens of a material that I have a lot of experience with. The lab I used to work in primarily used the synthetic polymer polyethylene glycol (PEG). It's a great material because of its versatility. Its material properties are highly controllable and can be tuned to mimic the ECM, making it ideal for tissue engineering scaffolds [3]. Let's say we are engineering a hypothetical soft tissue utilizing an acellular PEG hydrogel. On its own, without manipulating it somehow, PEG is relatively inert when placed in the body, and not much will happen. The body very well may ignore it regardless of where you put it; we would say there is no biocompatibility.
We may see some interaction if we add some adhesion molecules, which allow cells to adhere, migrate, and then place the scaffold into a biological system. Some cells may adhere just because it is thermodynamically favorable. Still, if we remember what I mentioned last time, cells often don't know what to do without some direction. Some might migrate into the scaffold just out of pure random chance. So, we might consider this a system with a higher degree of biocompatibility but still relatively low. Suppose we add chemical cues that signal the cells to migrate or incorporate a porous or mechanical gradient into the scaffold. In that case, we can then perhaps influence the cells at our hypothetical gradient to invade and migrate into a direction that we want, again increasing the overall degree of biocompatibility of the system.
This, of course, assumes that the cells are physically able to migrate through the scaffold. Remembering that these scaffolds are a network of fibers, they have empty spaces that the cells can physically travel through. However, suppose the matrix is too dense. In that case, the cells may not be able to physically fit through the fiber network, meaning that biocompatibility is low or nonexistent. This is why finding a good middle ground between molecular weight, cross-linking density, polymer type, and the mechanical properties can be challenging.
Let's now assume that the scaffold has the correct adhesion molecules, cues for migration, and the porosity is adequate. Let us say that the mechanical stiffnesses of the matrix are comparable to the native tissue. After implantation, the cells migrate into the scaffold and begin repopulating it. In this instance, let's say that everything is as it should be, except for the scaffold degradation rates. Ideally, the degradation rate should be proportional to the rate at which the cells deposit new ECM materials, which will ensure that the scaffold maintains its structural integrity and its bulk mechanical properties will not change. As I said in the previous article if the scaffold degrades too quickly, the whole implant may fall apart [8]. Conversely, if the scaffold degrades too slowly, it may trigger an aggressive immune response [5]. In both of these cases, biocompatibility is not observed. But we should be aware of the time-dependent nature in this case: it was not until it was time for the scaffold to degrade that problems came about, and not immediately after implantation.
Though there are countless more hypotheticals that we can entertain, there is one more that I want to go through. Let's say our implant all of the characteristics from the last example, and the scaffold degrades at a proportional rate of ECM deposition. Let's focus on the degraded scaffold components. One role of neutrophils and macrophages (two types of immune cells) is to clean up the remains of materials and other things that litter the body—not unlike a janitor picking up litter from the floor. They do this through a process known as phagocytosis, which can be thought of as cellular eating (Figure 5).

As a general rule, if a particle is less than 5 μm along its largest dimension, the immune cell will be able to phagocytose the particle. However, when particles are larger, a slightly different event occurs called frustrated phagocytosis. In this instance, the immune cell secretes enzymes to try and digest the particle and break it into a smaller size, so it can adequately devour the material. In cases with non-degradable materials in particular, though it can occur with degradable polymers that have not degraded sufficiently, the immune cell will struggle (hence the name "frustrated") to consume the material. It will continue to secrete more enzymes which can inflame tissue. Long-term this can potentially lead to chronic inflammation [5]. Therefore, even if your scaffold's other specifications and characteristics are ideal, ensuring that the body can adequately resorb the implanted material and not just degrade it at some specific rate is another time-dependent consideration that impacts biocompatibility.
There are many more hypotheticals that we could explore. Still, I hope that this was more than sufficient and made it clear that biocompatibility is not a material characteristic, but an aspect of the material-host system.
Series Conclusion
I want to thank all of you who managed to make it all the way to the end of this very long series. When I first considered writing this, I was not intended for it to be as long as it ended up being. We covered a lot of very complicated concepts, and I hope that I articulated them in a way that made them easy to understand. Part of the difficulty of explaining biomedical engineering concepts is that a lot of foundational material needs to be established before the more particular cases of the discipline can be discussed. Moreover, it pulls from biology, physiology, physics, chemistry, engineering, and advanced mathematics; often, people will have experience in only one of these areas. So to ensure that these concepts are as intelligible as possible to as many people as possible, I believe it's necessary to start at the foundation and work our way forward. There are still many, many things that I did not talk about. Maybe in the future, if the time calls for it, I can return and go through these things we skipped over.
My ultimate hope is that these four articles demystified many of the fundamentals that Foregen's work is built upon. Also, I hope those of you who took the time to read this series learned some new and exciting things; I know it probably was not the easiest, but I am genuinely grateful for those who made it all of the way through. Undoubtedly some sections may be confusing or unclear. If anyone has any specific questions or if something does not make sense, do not hesitate to reach out, and I'll do my best to clear things up.
Thank you again for all of your support, as we could not do any of what we do without it.
Take care.
References
- Call, M.K., and P.A. Tsonis, “Vertebrate Limb Regeneration,” in I. V. Yannas, ed., , “Regenerative Medicine I: Theories, Models and Methods,” Springer, Berlin, Heidelberg, Berlin, Heidelberg, pp. 67–81 (2005).
- Harries, R., J. Torkington, and K. Harding, “Advances in Acellular Extracellular Matrices (ECM) for Wound Healing,” in M. Z. Albanna and J. H. Holmes, eds., , “Skin Tissue Engineering and Regenerative Medicine,” Academic Press, London, UK, pp. 125–143 (2016).
- Papavasiliou, G., S. Sokic, and M. V. Turturro, “Synthetic PEG Hydrogels as Extracellular Matrix Mimics for Tissue Engineering Applications,” in R. Sammour, ed., , “Biotechnology - Molecular Studies and Novel Applications for Improved Quality of Human Life,” InTech, , pp. 111–134 (2012).
- Schopenhauer, A., “Über die vierfache Wurzel des Satzes vom zureichenden Grunde [On the Fourfold Root of the Principle of Sufficient Reason],” in D. E. Cartwright, E. E. Erdmann and C. Janaway, eds., , “On the Fourfold Root of the Principle of Sufficient Reason and Other Writings,” Cambridge University Press, Cambridge, UK, pp. 1–197 (2012).
- Temenoff, J.S., and A.G. Mikos, “Biomaterial Implantation and Acute Inflammation,” “Biomaterials: The Intersection of Biology and Materials Science,” Pearson Education, Inc., Upper Saddle River, NJ, pp. 369–384 (2008).
- Temenoff, J.S., and A.G. Mikos, “Wound Healing and the Presence of Biomaterials,” “Biomaterials: The Intersection of Biology and Materials Science,” Pearson Education, Inc., Upper Saddle River, NJ, pp. 385–402 (2008).
- Temenoff, J.S., and A.G. Mikos, “Materials for Biomedical Applications,” “Biomaterials: The Intersection of Biology and Materials Science,” Pearson Education, Inc., Upper Saddle River, NJ, pp. 1–31 (2008).
- Temenoff, J.S., and A.G. Mikos, “Biomaterial Degradation,” “Biomaterials: The Intersection of Biology and Materials Science,” Pearson Education, Inc., Upper Saddle River, NJ, pp. 177–204 (2008).
- Williams, D.F., “On the mechanisms of biocompatibility,” Biomaterials, 29 (20), pp. 2941–2953 (2008).
- Williams, D.F., “There is no such thing as a biocompatible material,” Biomaterials, 35 (38), pp. 10009–10014 (2014).
- Yannas, I. V., “Facts and Theories of Induced Organ Regeneration,” in I. V. Yannas, ed., , “Regenerative Medicine I: Theories, Models and Methods,” Springer, Berlin, Heidelberg, Berlin, Heidelberg, pp. 1–38 (2005).